“One of the hazards associated with underwater diving is decompression sickness (DCS), caused by uncontrolled release of gas from tissues during or after surfacing.”
Diving is a popular recreational pastime as well as an activity with numerous practical applications in the scientific, commercial, military and exploration realms. While diving can be done safely, it is essential for all divers — no matter what their reason for diving — to appreciate that the underwater environment is unforgiving. Problems may arise during a dive due to insufficient medical or physical fitness, improper use of equipment or inadequate management of the high-pressure environment.
One of the hazards associated with the pressurized underwater setting is decompression sickness (DCS), a condition also known as “the bends.” This chapter explains the basics of DCS, while subsequent chapters provide details regarding its manifestation and management, risk factors that may predispose you to the condition and preventive steps that you can take to minimize your chance of developing it.
In this chapter, you’ll learn about:
The Physiological Mechanisms of DCS
Tissue Tension
When a diver is exposed to an environment of elevated pressure, inert gases (nitrogen, for example) accumulate in tissues. The deeper a dive is, the faster the body’s absorption, or “uptake,” of such gases. When the diver ascends, the drive is reversed, and gas leaves tissues. A diver’s ascent must be controlled to allow for an orderly elimination, or “washout,” of the accumulated gas. A slow ascent, conducted either continuously or in stages, usually allows for safe decompression, whereas a too rapid ascent following gas accumulation can sometimes result in DCS.
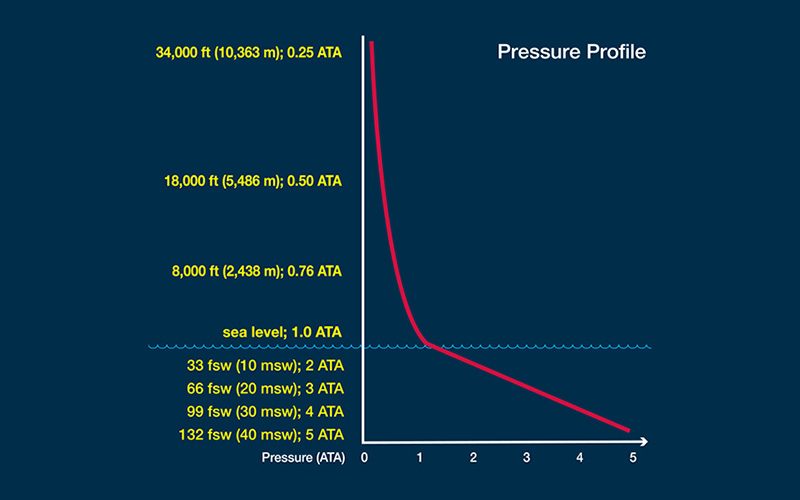
The concentration, or “tension,” of dissolved inert gas within your body’s tissues is a function of ambient pressure — that is, the pressure of the environment surrounding you at any given time. The inert gases that are not used in your body’s metabolic reactions normally exist in equilibrium with your ambient environment — in the same concentration as in the air around you. Tissues under such conditions are described as “saturated.” Minor pressure changes, such as those created by shifting weather conditions, produce minor pressure variations in atmospheric gases that are then matched by pressure changes in the gases in the body’s tissues. When a pressure difference, or “gradient,” is created, molecules from the area of higher concentration flow toward the area of lower concentration until balance is re-established. Since all of us constantly experience minor changes and corrections of this nature, the gas tension in our bodies is in a state of dynamic, rather than static, equilibrium — even before diving is added to the equation.
Pressure
The diving environment puts a significant additional burden on this adaptive mechanism. Here’s why: Pressure is measured using a unit known as an “atmosphere.” There is no actual physical boundary between the Earth’s atmosphere and space, but the atmosphere is often considered to extend 62 miles (100 kilometers) from sea level to the edge of outer space. The pressure produced by this entire column of gas acting at sea level is one atmosphere, equal to 14.7 pounds per square inch (psi) or 101.3 kilopascals (kPa). By comparison, the change in pressure underwater increases by one atmosphere for every 33 feet of saltwater and every 34 feet of freshwater. As a result, any variation you experience in surface atmospheric pressure is extremely modest compared with the variation in pressure you can undergo when you travel vertically underwater; this can create huge gradients in the uptake of gases during your descent and in their elimination during your ascent.
Gas Exchange
Your lungs serve as the primary connection between your body and the environment in which you are situated at any given time. When you expose yourself to increased pressure underwater, the gas in your lungs is compressed. This creates a gradient from your lungs to your bloodstream and, subsequently, from your bloodstream into your tissues as they are perfused, or supplied, with oxygenated blood. Your tissues will take up inert gas until the gradient is eliminated, an effective state of equilibrium, or saturation, with the surrounding environmental pressure. It takes a long exposure for complete saturation to be reached, but once reached, staying longer does not further increase gas uptake or the required decompression.
Predicting Gas Uptake and Elimination
Tissue Compartments
This natural physiological mechanism can be predicted by a series of mathematical algorithms based on “half-time compartments,” which approximate the exponential uptake and elimination patterns expected in various types of perfused tissues. The key to these algorithms is that different parts of the body take up and eliminate inert gases at differing rates — for example, blood is considered a “fast compartment” and bone a “slow compartment.” (The term “compartment” is not meant as an exact referent for these tissues but, rather, as a mathematical construct to estimate what happens in various parts of the body.)
The fastest tissues are the lungs, which achieve equilibrium almost instantly. Blood follows in speed, then the brain. The slowest tissues are those that are relatively poorly perfused, such as ligaments and cartilage, or those that have a high capacity for inert gas uptake, such as fat in poorly perfused areas. The reason for using a mathematical algorithm to estimate tissue status is that it is not yet practical to directly measure uptake or elimination in specific tissues.
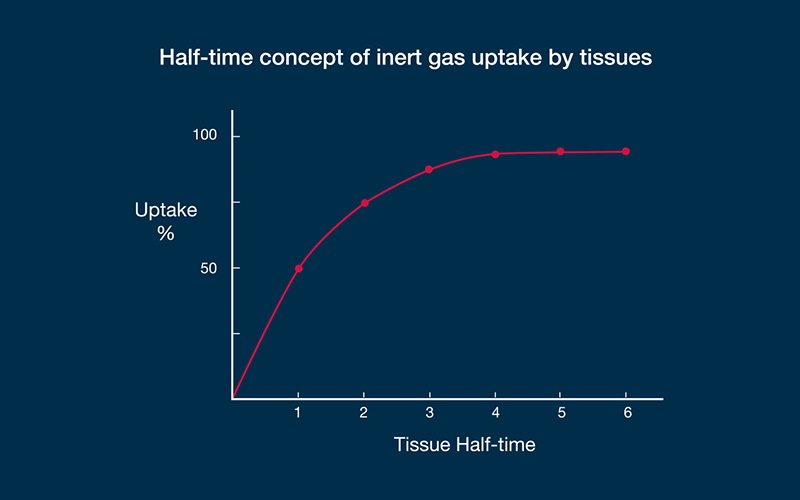
An example may demonstrate how the algorithms work. Let us imagine a diver who has been instantly displaced from the surface to a fixed depth — effectively, a fixed pressure — and let us say that in this particular dive scenario, a fast compartment has a half-time of five minutes. In such a case, the first five minutes of exposure to the higher pressure would result in sufficient inert gas uptake to eliminate half of the difference produced by the pressure gradient (50 percent, in other words); this is the steepest portion of the uptake curve. The second five-minute period would eliminate half of the remaining difference (another 25 percent). The third five-minute period would eliminate half of the remaining difference (12.5 percent); the fourth, 6.25 percent; the fifth, 3.125 percent; and so on. This exponential pattern means that the rate of change becomes progressively slower as the magnitude of the difference decreases. The example described a fast compartment; half-times for slow compartments have been computed in some algorithms out to almost 500 minutes. In decompression theory, the absolute difference in pressure is immaterial — the same half-time construct applies to any gradient. With no additional influences on the process, equilibration, or saturation, would be achieved in a period equal to about six half-times. As gas dissolves in the tissue, the difference between the external pressure and internal pressure decreases, reducing the driving force.
Most dives do not last long enough for the diver to reach saturation — these are known as “bounce dives.” During such exposures, the inflow gradient exists throughout the descent and bottom phase of the dive, which causes continued uptake of inert gases, certainly in the body’s slow compartments and probably in intermediate compartments. When the diver starts to ascend, and the ambient pressure starts to drop, the gradient begins to reverse — first in fast compartments and then in progressively slower compartments.
Degree of Supersaturation
Effectively, during and after surfacing, most of a diver’s tissues will be supersaturated in comparison with the ambient pressure. If the degree of supersaturation is modest, inert gases can travel in an orderly manner from the body’s peripheral tissues into the blood and then to the lungs, from where they can be exhaled to the atmosphere. But if the degree of supersaturation is too great, the elimination of inert gases becomes disorderly. In this case, gas bubbles can form in the tissues of the diver’s body.
Bubble formation does not always cause problems, but the higher the gradient, or degree of supersaturation, the greater the likelihood that signs and symptoms of DCS can occur.

It is a dangerous misconception that measurable bubbles form after all dives and are of no importance. But at the same time, it is a misconception that bubbles visualized in the blood stream in and of themselves signal DCS. The formation of gas bubbles during decompression represents a stress greater than is optimal and may lead to DCS. It is best to follow conservative dive profiles to minimize the likelihood of bubble formation. The greatest difficulty is in knowing what counts as “conservative,” since most divers have never been monitored for bubbles, and uptake and elimination is altered by a number of factors in addition to the pressure-time profile.
The half-time compartment calculations are used to generate exposure-limit predictions for a range of hypothetical compartments. In paper or plastic form these are known as “dive tables.” Modern dive computers allow for much more flexible guidance since they are able to continuously monitor the pressure-time profile and simultaneously compute the status of a variety of theoretical tissue compartments. But in reality, the picture is much more complex. Gas exchange is influenced by more than just the pressure-time profile. So while it is important for divers to understand the concepts behind calculating half-time compartments, divers must also keep in mind that a wide range of factors can influence gas uptake and elimination and effectively alter decompression risk. Thus the onus is on the diver not to rely too heavily on a table or device for safety.